The origin of fractures in rock is not always obvious
When she sees fractures in outcrop, a geologist might assume that these fractures are the consequences of a protracted loading at depth due to burial, uplift, pore pressure changes, or to a regional tectonic deformation event (e.g. Engelder, 1985). Some or all of these processes can lead to fractures, and the exact loading path can in many cases be hard to unravel by examining the fractures alone (e.g., Laubach et al., 2019). In the case of platform carbonates however, the fracture history may start far earlier in the rock’s history than many structural geologists might suspect. In many cases, platform carbonates—especially if the platforms have steep and unstable margins—become fractured during or shortly after deposition before they are even buried. This kind of deformation is referred to as syndepositional fracturing, meaning that the fractures are broadly contemporaneous with deposition. These early features can be faults or opening-mode fractures. Commonly they appear as large-aperture, steeply dipping, opening-mode fractures with fill or cross-cutting relationships that indicate their timing during deposition, and an alignment consistent with loads caused by the shape of the platform margin (Frost & Kerans, 2010). Such fractures have also been referred to as “Neptunian Dikes” (Playford, 1984; Smart et al., 1988).
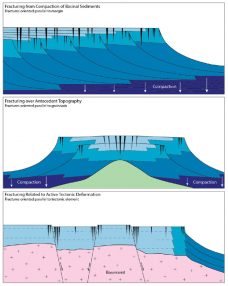
Figure 1. Three drivers of syndepositional fracturing (Simon 2014, modified from Frost, 2007, and Frost & Kerans 2010.
How
Many sediments require considerable burial and heating to consolidate into brittle rocks. Compaction and the precipitation of minerals are two processes by which such lithification is accomplished. For the conversion of sands into sandstones, for example, deep (several kilometers) or protracted burial and heating might be needed to make really brittle rocks (e.g., Taylor et al., 2010; Laubach et al., 2019, their figure 15). In the absence of such a burial history, for example, in the Texas Hill Country, some silica-rich clastic ‘formations’ of Cretaceous age are still unconsolidated ‘sands’. Carbonate materials however differ due to being constituted of extremely reactive minerals. This can result in “geologically instantaneous” (Grammar et al, 1999) cementation and embrittlement of the carbonate material, making it susceptible to fracturing during or shortly after deposition (Frost & Kerans, 2010). Certain substrates in particular, such as mud-rich carbonate facies tend to precipitate calcite cements much earlier than others (LaBruna, 2020).
There are three main drivers of syndepositional fracturing (e.g., Frost, 2007) (Figure 1). The first is gravitational instability and substrate compaction. Deformation is initiated by extensional loads associated with the over steepening of carbonate platform escarpments, or from down-to-the-basin tilting of the entire platform (Frost & Kerans 2010). Progradational platform systems with a high lateral growth rate are the most susceptible to this type of gravitational collapse (Resor & Flodin, 2010; Frost, 2007; Narr, 2008). This type of deformation can result in high fracture abundance (intensity) at the platform-slope margin where bending strains are highest with lower fracture intensity as one moves towards the platform interior (Frost & Kerans, 2010). Such fractures are generally oriented parallel to the carbonate platform margin (Kosa & Hunt, 2005; Hunt et al., 2002; Narr, 2008).
A second driver is differential compaction over pre-existing (antecedent) topography. Deformation can result from the flexure of early cemented carbonate strata over an ancient topographic high sitting below the surface. Fractures may occur as a cluster of closely spaced fractures (a swarm; Laubach et al. 2018) aligned parallel to the crest of the buried topographic element (Frost & Kerans, 2010).
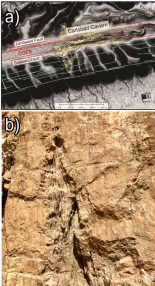
Figure 2. Syndepositional effects. (a) Carlsbad cavern cave system formed along syndepositional platform faults, West Texas, from Mathis, (2014). (b) Karst features in a likely syndepositional fault in Bronzo Quarry, South of France (Photograph: B. Rysak, 2019).
The third mechanism for early fracturing is local or regional tectonics combined with elevated pore fluid pressure manifest in opening-mode fractures, faults or folds. Faults and folds can in principle form at any time in a rock’s history from various causes, from plate-scale deformation to diverse highly localized causes. If such processes are active while carbonate materials are experiencing early embrittlement, they can be the cause of synsedimentary fractures. These fractures are expected to be oriented parallel to active faults and folds and within fault damage zones (Frost & Kerans, 2010; Miller et al., 2007) but in fact the range of orientations and spatial arrangements from such causes can be highly diverse (e.g., Hancock, 1985). Consequently, identifying results of this third mechanism can be challenging and usually requires evidence from the fractures that they formed early but not as a consequence of either of the first two mechanisms; a tall order (Simon, 2014).
In nature, all of these three drivers for early fracture may be active at the same time (e.g., Frost & Kerans, 2010). All together these driving mechanisms show that forces outside the depositional system such as regional tectonics or elevated pore fluid pressure are not always necessary to create extensive brittle deformation (Lamarche et al., 2012; Frost & Kerans, 2010). This diversity of potential causes for fractures complicates the search for fracture origins.
So what?
And now for the final question, why are early formed fractures important? To that, there are many answers.
Syndepositional fractures tend to be preferentially reactivated throughout the evolution of a platform and can evolve into, for example, extensional faults (Simon, 2014) and thus influence the overall mechanical strength and deformation pattern of a platform during subsequent events. Moreover, if early formed, these areas of weakness and planes of failure can modify subsequent facies distribution and sedimentary patterns of younger sediments, as well as cause mass-wasting of the platform edges (Frost, 2007; Rush & Kerans, 2010). Evidence of these proce
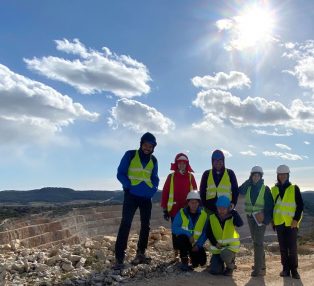
Bethany Rysak (second from left) during field work, France, 2019
sses has been seen in places like the Capitan reef on the New Mexico side of the Permian Basin. Resulting extensional faults separating large blocks is in marked contrast to previous facies correlations in the area that assume stratigraphic continuity (e.g., Dutton et al., 2005).
Early fractures and faults, if they remain open, can also be fluid flow conduits from the earliest phase of platform growth through to eventual burial and uplift. Thus syndepositional deformation features (opening-mode fractures and faults) may be primary fluid conduits through “otherwise impermeable strata” (Simon, 2014), facilitating the movements of fluids such as water or hydrocarbons, and aiding in localized diagenesis of the host rock (La Bruna, 2020; Hunt et al., 2002). Once uplifted they can also be the site for largescale near-surface karst development (Figure 2) (Mathisen, 2014; Frost & Kerans, 2010; Lamarche et al., 2012) or they can localize deep-seated dissolution (Baques et al., 2020).
In addition, the nature of the formation of syndepositional fractures creates fracture patterns that are not random, meaning that their location may be predictable (Hunt et al., 2002; Kosa & Hunt 2005; Frost, 2007, Frost & Kerans, 2010). By knowing the location of platform margins, buried topographic highs, embrittlement-prone lithofacies distributions, and subsequent folds and faults, one can accurately determine probable high-density fracture areas. This is hugely significant for exploration and engineering operations like oil and gas well placement and aquifer analysis. In an otherwise impermeable rock, the location of syndepositional faults and fractures may directly control the areas of greatest fluid production and thus should be accounted for in reservoir development (Frost & Kerans, 2010). In places like the Tengiz Field in Kazakhstan, syndepositional fractures were found to be “the most important for reservoir productivity” (Narr, 2008).
Closing
Syndepositional fractures can be seen today in outcrops from the South of France to West Texas to the coasts of Australia. These features, while perhaps underappreciated, have played a huge role in overall regional structure due to their early initiation. Syndepositional fractures can control fluid flow, fluid trapping (reservoir formation), diagenesis, karst development, and subsequent deformation. So next time you encounter a fault or fracture in an outcrop of carbonate rock, don’t immediately write it off as a purely ‘structural’ feature. Give those fractures a second look. There’s a good chance part of the rock’s earliest geologic history may be locked up in that single feature.
References
Baqués, V., Ukar, E., Laubach, S.E., Forstner, S.R., Fall, A., 2020. Fracture, dissolution, and cementation events in Ordovician carbonate reservoirs, Tarim basin, NW China. Geofluids, v. 2020, Article ID 9037429, 28 p. doi: 10.1155/2020/9037429
Dutton, S.P., Kim, E.M., Broadhead, R.F., Raatz, W.D., Breton, C.L., Ruppel, S.C., Kerans, C., 2005. Play analysis and leading-edge oil-reservoir development methods in the Permian basin: Increased recovery through advanced technologies. AAPG Bulletin, 89(5), 553-576.
Engelder, T., 1985. Loading paths to joint propagation during a tectonic cycle: an example from the Appalachian Plateau, USA. Journal of Structural Geology, 7(3-4), 459-476.
Frost, E.L., 2007. Facies heterogeneity, platform architecture and fracture patterns of the Devonian reef complexes. PhD dissertation, The University of Texas at Austin.
Frost, E.L., Kerans, C., 2010. Controls on syndepositional fracture patterns, Devonian reef complexes, Canning Basin, Western Australia: Journal of Structural Geology 32, 1231–1249.
Grammer, G.M., Crescini, C.M., McNeill, D.F., Taylor, L.H., 1999. Quantifying rates of syndepositional marine cementation in deeper platform environments – new insight into a fundamental process: Journal of Sedimentary Research 69, 202–207.
Hancock, P.L., 1985. Brittle microtectonics: principles and practice. Journal of Structural Geology, 7(3-4), 437-457.
Hunt, D.W., Fitchen, W.M., Kosa, E., 2002. Syndepositional deformation of the Permian Capitan reef carbonate platform, Guadalupe Mountains, New Mexico, USA. Sedimentary Geology 154, 89–126.
Kosa, E., Hunt, D.W., 2005. Growth of syndepositional faults in carbonate strata: Upper Permian Capitan Platform, New Mexico, USA. Journal of Structural Geology 27, 1069–1094.
La Bruna, V., Lamarche, J., Agosta, F., Rustichelli, A., Giuffrida, A., Salardon, R., Marie, L., 2020. Structural diagenesis of shallow platform carbonates: Role of early embrittlement on fracture setting and distribution, case study of Monte Alpi (Southern Apennines, Italy). Journal of Structural Geology 131, 17 p.
Lamarche, J., Lavenu, A.P.C., Gauthier, B.D.M., Guglielmi, Y., Jayet, O., 2012. Relationships between fracture patterns, geodynamics and mechanical stratigraphy in carbonates (South-East Basin, France). Tectonophysics, 581, 231–245.
Laubach, S.E., Lander, R.H., Criscenti, L.J., et al., 2019. The role of chemistry in fracture pattern development and opportunities to advance interpretations of geological materials. Reviews of Geophysics, 57 (3), 1065-1111. doi:10.1029/2019RG000671
Laubach, S.E., Lamarche, J., Gauthier, B.D.M., Dunne, W.M., Sanderson, D.J., 2018. Spatial arrangement of faults and opening-mode fractures. Journal of Structural Geology 108, 2-15. doi.org.10.1016/j.jsg.2017.08.008
Marrett, R., Laubach, S.E., 2001. Fracturing during Burial Diagenesis. The University of Texas at Austin, Guidebook 28. Bureau of Economic Geology, 109–123.
Mathisen, M., 2014. Spatial and Temporal Evolution of the Cave Graben Fault System, Guadalupe Mountains, New Mexico: The University of Texas at Austin.
Miller, J.M., Nelson, E.P., Hitzman, M., Muccilli, P., Hall, W.D.M., 2007. Orthorhombic fault-fracture patterns and non-plane strain in a synthetic transfer zone during rifting: Lennard shelf, Canning basin, Western Australia: Journal of Structural Geology 29, 1002–1021.
Narr, W., Fischer, D.J. Harris, P.M. Heidrick, T. Robertson, B.T., Payrazyan, K., 2008. Understanding and Predicting Fractures at Tengiz – A Giant, Naturally Fractured Reservoir in the Caspian Basin of Kazakhstan.
Playford, P.E., 1984. Platform-margin and marginal-slope relationships in Devonian reef complexes of the Canning Basin, in: Purcell, P.G., (Ed.), The Canning Basin W.A: Proceedings of the Geological Society of Australia/Petroleum Exploration.
Price, N.J., 1966. Fault and Joint Development in Brittle and Semi-brittle Rock. Pergamon Press, Oxford.
Resor, P.G., Flodin, E.A., 2010. Forward modeling of synsedimentary deformation associated with a prograding steep rimmed carbonate margin: Journal of Structural Geology 32, 1187-1200.
Rush, J., Kerans, C., 2010. Stratigraphic response across a structurally dynamic shelf: the latest Guadalupian composite sequence at Walnut Canyon, New Mexico, USA. Journal of Sedimentary Research, 80, 808-828.
Simon, R., 2014. Syndepositional Fault Control on Dolomitization of a Steep-Walled Carbonate Platform Margin, Yates Formation, Rattlesnake Canyon, New Mexico: The University of Texas at Austin.
Smart P.L., Palmer R.J., Whitaker F., Paul Wright V., 1988. Neptunian dikes and fissure fills: an overview. In: James N.P., Choquette P.W. (eds) Paleokarst. Springer, New York, NY.
Taylor, T.R., Giles, M.R., Hathon, L.A., Diggs, T.N., Braunsdorf, N.R., Birbiglia, G.V., Kittridge, M.G., Macaulay, C.I. Espejo, I.S., 2010. Sandstone diagenesis and reservoir quality prediction: Models, myths, and reality. AAPG Bulletin, 94(8), 1093-1132.
About the Author
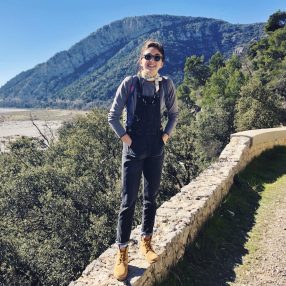
Bethany Rysak during field work in France, 2019
Bethany Rysak is a Master’s student at The University of Texas. Her research is on hydraulic fractures in shale.
© 2024 Jackson School of Geosciences, The University of Texas at Austin