Carbonate rocks are fascinating complex rocks that have challenged geologists for centuries. Natural fractures are a crucial part of this complexity.
A huge amount of the world’s oil and gas reserves are stored in carbonate reservoirs. A fairly robust review estimated volumes of 50 to 60% (e.g., Garland et al. 2012). Examples of large oil fields in carbonate rocks are Ghawar Field in Middle East, Lula Field in Brazil, and Kashagan Field in Kazakhstan.
There are many challenges to fracture characterization in all rock types (e.g., Laubach et al. 2019). But the unique high lithological heterogeneity and diagenetic reactivity characteristics of carbonate rocks impose some peculiar challenges to standard fracture characterization workflows.
Many low-matrix-porosity hydrocarbon reservoirs are productive because permeability, and sometimes porosity, are controlled by opening-mode fractures and faults. Fractures are suspected to play a significant role in many reservoirs in carbonate rocks (e.g., Garland et al. 2012). Where naturally fractured, carbonate rocks may show increased permeability and yield outstanding reservoir productivity (e.g., Narr et al. 2006).
Because of the substantial impact fractures may have on reservoir productivity, the subject of fractured reservoirs has become one of industry and academia’s hottest topics for technology development and research investment.
In this summary, I touch on ten challenges in naturally fractured carbonate rocks, discuss some tricky petrologic aspects in carbonates, and recall some literature examples that take these topics further.
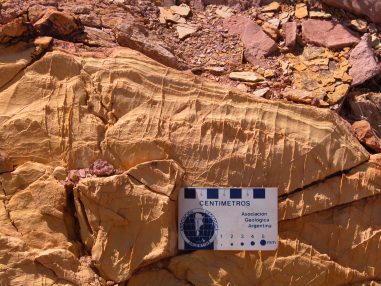
Early fractures, Argentina
Syn-sedimentary deformation: the early brittle history
Carbonate deposits can possess structural complexity caused by widespread syn-sedimentary deformation. Carbonate deposits are unique in that they may become rocks during or shortly after deposition. This means that compared to other rocks of the same age, carbonates potentially experience a longer history of brittle deformation.
Carbonate materials can be cemented early in their depositional history, in some cases virtually at deposition. For this reason, some early cemented carbonate layers may be prone to syn-sedimentary faulting and fracturing (e.g., Enos and Sawatsky 1981). Some syn-sedimentary fractures may be related to differential compaction or to the topography of the depositional system (fractures aligned with reef fronts are well known). In most cases, fractures related to syn-sedimentary processes have sediment fills, although sediment can infiltrate much later and is a common feature in karst systems that may reflect much later uplift into vadose conditions.
Another manifestation of syn-sedimentary deformation includes carbonate materials deposited directly from vents in fault zones, like tufas and travertines (e.g., Benson 1994). These types of carbonates may be adjacent to and above fault zones and deposits may be subsequently deformed by fault movement.
Among the challenges of assessing syn-sedimentary deformation in the subsurface are identifying syn-sedimentary processes that have attributes that may overlap those of structures formed at depth, and extrapolating patterns away from well control.
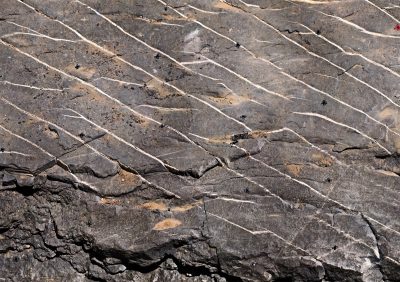
Fracture patterns, Oman.
Depositional heterogeneity: Vagaries of depositional and mechanical stratigraphy
Carbonate rocks can have wide range of compositions and primary depositional fabrics (e.g., Scholle and Ulmer-Scholle 2003; Flugel 2010). Corals, gastropods, peloids and ooids are some of the different grain types that can be present. This wide range of different grains and depositional fabrics also might yield more mechanical heterogeneity for carbonate rocks (Durrast and Siegesmund 1999) compared, for example, to siliciclastic rocks.
This kind of mechanical heterogeneity can affect how fractures are distributed in the rock (Nelson and Serra 1995; Laubach et al. 2009). For instance, due to its rigid framework, coral reef deposits may show higher unconfined compressive strength (UCS) than interbedded ooid grainstones. If both rocks are subjected to similar deformation, this rheological difference can result in different fracture intensities, just because the response to deformation would be in accordance to the different mechanical properties of constituent rock types, even in the same formation or sequence.
Carbonates may also show abruptly varying mechanical layer thickness caused by features such as mounds or reefs. All these different fabrics, facies and architectures are a background framework for fracture distribution in carbonate rocks and may in some instances control fracture distribution.
However, some obvious depositional heterogeneities may have little or no impact on mechanical or fracture stratigraphy (e.g. Lavenu & Lamarche 2018). The differences in lithology may not amount to meaningful mechanical differences. Fracture patterns and fracture stratigraphy also depends in a fundamental way on leading history. So fractures may or may not reflect either type of mechanical heterogeneity. In fact, as Gillespie et al. (1999) inferred, what constitutes a meaningful mechanical heterogeneity may depend on confinement, other aspects of loading, possibly in ways that are not straightforward.
The subsurface challenge is to know which rock types and fabrics actually affect fracture distribution and how.
High reactivity: The eraser
The tendency for carbonate rocks to dissolve and recrystallize under near-surface and deep subsurface conditions is notable. Indeed, few carbonate rocks have properties that resemble those of recently deposited sediments. And some reservoirs in carbonate rocks reside in dissolution cavities that can be of considerable size. The mineralogy of most carbonate rocks is dominated by aragonite (initially), calcite and dolomite, plus minor phases including clay minerals (e.g., Scholle and Ulmer-Scholle 2003, Wright & Barnett 2015). Aragonite and high-magnesium calcite are two of the most reactive minerals, and usually are not preserved over geological time. When leached, aragonite grains may yield large pores or may be replaced by other more stable minerals (Flugel 2010). Low-magnesium calcite is the most common mineral, but it is also prone to dissolution or recrystallization depending on diagenetic and fluid flow history of the rock.
Fractures in carbonate rocks are also subject to dissolution and precipitation reactions and fracture fills may have mineralogical complexity (multiple phases) (Gale et al. 2010). Fracture cements may be affected by dissolution, recrystallization, and other alterations, complicating assessment of original cement presence, composition, or fabric. Recrystallization may blur fracture apertures if neo-formed crystal sizes are bigger than apertures.
High reactivity poses several challenges for subsurface fracture evaluation. Indeed, all of the rest of the challenges picked out below are to some extent the result of high reactivity. A less widely appreciated challenge is that high reactivity tends to erase the structural record, impeding reconstruction of the causes and timing of fracture formation. An example is the loss of distinctive textures in fracture cements (Ukar and Laubach 2016).
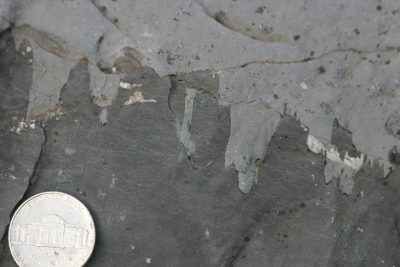
Stylolites, Mexico
Stylolites: interpreting pervasive anti-cracks
Stylolites are narrow (mm) tabular to irregularly shaped zones having serrate, tooth-like surface topology. They belong to a family of sub-planar defects caused by localized volume reduction (LVR), alongside compaction bands, localized negative-volume phase transitions, and dehydration structures. They are widely considered to have formed by shortening—commonly normal to bedding—driven by processes of pressure and chemical dissolution. Bed-normal compaction is a common way for stylolites to form in carbonate rocks. But under mild bed-parallel shortening they may form at a high angle to bedding (in which case they can be considered to be a kind of disjunctive spaced cleavage: Engelder and Marshak, 1985). Although stylolites are usually not classified as a type of fracture, they may be usefully thought as contraction fractures. They may propagate like fractures, as ‘anti-cracks’ (Fletcher and Pollard, 1981). More recent studies (Aharonov and Karcz 2019) have questioned the anti-crack model, claiming that some stylolite tips sometimes show tensile structures such as veins, suggesting a more complex stress state.
As carbonate rocks are highly susceptible to pressure solution, these rocks usually show many stylolite structures. Stylolites usually are composed of clay, oxides and organic matter (Flugel 2010). If exposed to dissolving fluids, stylolites may also become permeability enhanced structures or be turned into cave systems. Stylolites are common structures in carbonate rocks, and usually occur together with other types of fractures adding complexity to the fracture network.
Unraveling the structural history of carbonate rocks should take stylolites into account. A challenge, and an opportunity, is to weave the kinematic and relative timing information from stylolites into overall carbonate rock structural interpretations.
Preservation and interpretation of fluid inclusions
Valuable histories of burial and paleo-temperature are trapped in fluid inclusions, which are minute cavities within minerals. A recent published study (Fall and Bodnar 2018) investigated the precision of fluid inclusion assemblages (genetically related arrays of fluid inclusions) to constrain fluid temperatures. A measure of precision is the median range of homogenization temperatures in individual fluid inclusion assemblages, which Fall and Bodnar 2018 found to be as little as 2°C for assemblages in tight-gas (low-permeability) sandstone environments. This means that fluid inclusion assemblages can provide powerful evidence of temperature, salinity, and pressure history in some sedimentary rocks.
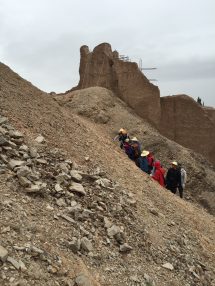
Fractured carbonate rocks, Tarim basin
There are several challenges however. Historically, fluid inclusion studies have paid inadequate attention to the petrographic context of the inclusions. A related problem is that some carbonate minerals, with current imaging methods, lack evidence of the textural relations needed to put fluid inclusions into proper context (Ukar and Laubach, 2016). Inclusions in carbonate minerals are also widely suspect because soft carbonate minerals can stretch or break, violating assumptions that allow trapping temperatures to be recovered.
With adequate microthermometry studies and burial control over the relative sequence of fluid inclusion assembly formation, it is possible to infer fracture age and opening rates for strong minerals like quartz, that are reliable hosts for pristine fluid inclusions (e.g. Fall et al. 2015; Laubach et al. 2016). Due to the natural instability of some carbonate mineral phases like aragonite and high magnesium calcite, fluid inclusions trapped in those minerals may be affected by neomorphism and thus can be reequilibrated (Goldstein 1986). When this happens, the fluid inclusion no longer accurately stores the primary inclusion composition and is usually reequilibrated to the neomorphism composition. Because of this, the more reliable fluid inclusions in carbonate rocks are often in low magnesium calcite, that are likely stable.
To fully exploit the valuable information trapped in fluid inclusion, the challenges of possibly poor preservation of inclusions, and cryptic textural context, will need to be overcome.
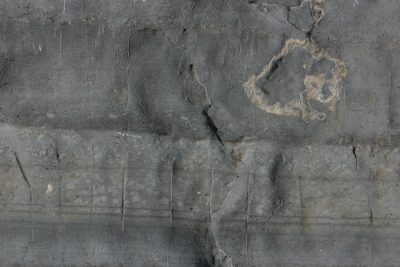
Fracture stratigraphy, Mexico
Understanding the complex mechanical evolution
A corollary to depositional heterogeneity is the potential in carbonate rocks for complex mechanical property evolution. However, rocks also mechanically evolve by processes beyond the depositional realm, such as diagenesis. Mostly because of sensitivity to diagenesis, including dissolution and precipitation reactions, coupled with heterogeneous and reactive starting materials, carbonate rocks tend to have complex mechanical evolution.
Progressive diagenesis results in both gradual and abrupt changes of the mechanical properties, and these mechanical properties continue to evolve with time (Laubach et al. 2009). For instance, a very porous loose grainstone may become a very tight dolostone prone to fracturing, after a dolomitization process. Sometimes the same rock unit may have different mechanical behaviors in different locations, simply because some parts of it have been affected in different ways by diagenesis or deformation. This kind of complexity makes it harder to define or at least extrapolate reliable mechanical units in carbonate rocks. Loading history will also play an important role in the final distribution of fractures.
An example of this challenge is the Austin Chalk, Texas. Although the Austin Chalk has a mostly constant tripartite mechanical stratigraphy (strong upper and lower chalk and weak middle chalk, owing to depositional differences in clay mineral distribution), the relationship between the mechanical and the fracture stratigraphy needs to be handled with great care. For example, tunnel exposures at depths of 30m show rocks mostly devoid of fractures in the same fracture-prone intervals that show abundant fractures in outcrops (Laubach et al. 2009). As expected, the upper and lower chalk are more fracture prone than the middle chalk in both tunnel and outcrop. But the absolute numbers of fractures in the two structural settings are drastically different. Outcrop fracture abundance measures are very poor guides to subsurface fracture abundance. This variation in Austin Chalk is a result of different deformation amounts affecting the same mechanical stratigraphy. The two structural settings have distinct fracture stratigraphy, even though a relatively constant mechanical stratigraphy is present. This example shows that mechanical and fracture stratigraphy are two different things.
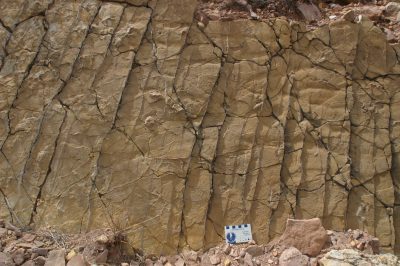
Fracture pattern, NW Argentina
Another example that drives home this point is from fractured carbonates described by Lavenu and Lamarche 2018. The Maiella/Murge mountains in Italy have outcrop fractures that are unbounded and freely cut bedding surfaces. Yet clear mechanical boundaries between beds, enhanced by stylolites, are seen nowadays. The fractures seem to ignore the clear mechanical boundaries. Why? The reason for this discrepancy is due to early development of fractures in a timeframe before the present mechanical stratigraphy was entirely set. Mechanical evolution in carbonates is affected by depositional heterogeneities, diagenesis, deformation distribution and timing. Even though there are cases in which fractures follow the mechanical stratigraphy, a more complex mechanical evolution may unattach fractures from this rock framework.
A challenge for fracture carbonate studies is to use this complexity in mechanical and fracture attribute evolution to produce more reliable attribute extrapolations and predictions. Since one of the biggest impediments to understanding opening-mode fractures is their inscrutable simplicity (i.e., Laubach et al. 2019), the challenge of mechanical complexity could be a great advantage to fracture analysis.
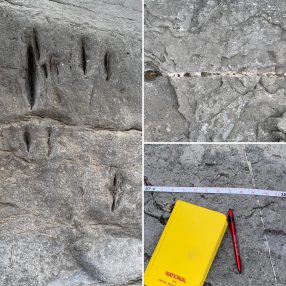
Solution enhanced and bridged fractures, central Texas
Karstification: lost news
Karstification or dissolution is also a characteristic process in carbonate rocks. Although karst (ancient, or paleokarst) is conventionally viewed as a near surface process occurring mostly when rocks are within the phreatic zone, deep-seated dissolution is well attested and is an important process in many deeply buried reservoirs.
The Ordovician Yijianfang Formation, from Tarim Basin, NW China is an example of deeply buried highly productive oil and gas reservoirs associated with large cavities (> 10m). Recent studies (Ukar et al. in press), have shown that dissolution occurred at depth and was caused by acidic, high temperature and Mg-rich fluids. This deep mesogenetic dissolution is associated with fractures and is the principal source of porosity and permeability for this reservoir.
Karstification can dissolve fracture walls and cements, and may enlarge kinematic apertures, making estimates of initial mechanical opening displacements unreliable. In advanced stages of karstification or deep-seated dissolution, fractures may be transformed into caves (Silva et al. 2017), making it harder to acquire any data on fracture kinematics, aperture, fillings or fluid inclusions where the cavities have developed.
A fundamental challenge in carbonate rock analysis is interpreting rocks that have been subjected to a process like dissolution that by its nature erases evidence.
Dolomitization: a common diagenetic product
Dolomite is a calcium/magnesium carbonate mineral. It is usually a product of diagenesis via dolomitization processes (e.g. Flugel 2010; Lucia and Major 1994). The most common model of dolomitization is called seepage reflux. This model suggests that dolomitization initiates where magnesium rich brines infiltrates the limestone. Once it replaces primary mineralogy, usually it destroys original fabric, making identification of the previous protolith difficult.
Some studies positively correlate dolomite content with fracture intensities, suggesting that understanding dolomitization framework may be important to predict fracture distribution. Some dolomitized rocks are directly linked with faults and fractures (as in the cover art for Garland et al. 2012). A challenge will be unraveling these linked chemical and mechanical processes, to be able to predict when dolomitization is controlling fracture stratigraphy or not.
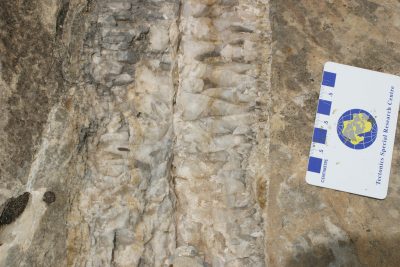
Calcite-filled fracture, Utah
Hydrothermalism: when deep fluids matter
As already told, carbonates like travertines and tufas may originate in active fault systems. Sometimes, these fault systems are in very active thermal sites. Hydrothermal diagenesis occurs when fluids are introduced via faults or other conduits into a host formation at a temperature that exceeds the ambient temperature of that formation by at least 5°C (Smith and Davies 2006). Because of that, carbonates formed in this type of environment are prone to be affected by hydrothermal diagenesis, which may precipitate or dissolve carbonate minerals, and very commonly dolomitize carbonate rocks. High pressure fluids, driven by hydrothermal processes, may cause hydraulic brecciation, forming nontectonic fractures (Ruggieri 1999). Examples of this can be seen in the Tarim Basin in China which shows dissolution caused by hydrothermal fluids that rose up through faults (Liu et al. 2017).
The challenge is to identify hydrothermal processes that may overcome tectonic features.
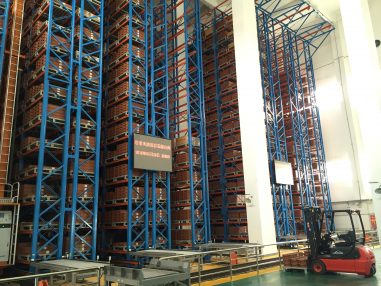
Carbonate rock core, China
The challenges of data acquisition
Fracture data acquisition is a considerable challenge in all rock types. Fractures may be widely spaced, they are generally narrow relative to sampling volumes (such as wellbores), and many are impossible to detect by indirect geophysical methods. But the challenges of heterogeneity and reactivity in carbonate rocks can make it harder to acquire data in carbonate formations. For example, collecting aperture-size data along 1D lines of observation (scanlines) yields mostly unambiguous results for siliciclastic rocks. But for many carbonate rocks complexities caused by syn-sedimentary deformation, dissolution, recrystallization, and dolomitization can complicate data acquisition. Some of these processes, for example, those merely affecting the outcrop, many not be relevant to understanding the subsurface. This ambiguity can result in an overall increase in data uncertainty, which may affect correct identification of size or spatial scaling patterns. The challenge is particularly insidious for reservoirs having extensive dissolution, because even if fractures are successfully encountered by the wellbore, dissolution enhanced apertures or cavities yield little information that can be used to extrapolate their size and character away from the wellbore.
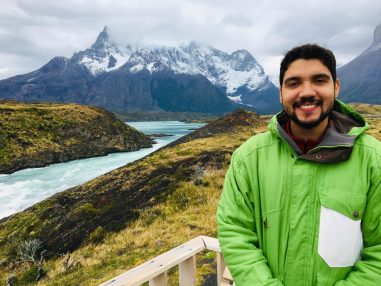
Rodrigo Correa
Author
Rodrigo Correa is a PhD student from The University of Texas at Austin, associated with the FRAC group. He graduated as a geology BSc. at the Universidade Federal do Rio de Janeiro in 2009 and geomechanics MSc. at Pontificia Universidade Catolica do Rio de Janeiro in 2016. He has been working for Petrobras, the Brazilian national oil company since 2010. In 2014, he joined Petrobras reservoir development team for Santos Basin Pre-salt fields and was responsible for the reservoir geology of diverse assets in carbonate reservoirs. He joined The University of Texas at Austin in 2019 as a full-time PhD student sponsored by Petrobras. His research is focused on fractured carbonates.
Acknowledgments
I am grateful for the constructive discussions and suggestions made by Bethany Rysak and Stephen E. Laubach.
References cited
Aharonov, E., & Karcz, Z. (2019). How stylolite tips crack rocks. Journal of Structural Geology, 118, 299-307.
Benson, L. (1994). Carbonate deposition, Pyramid Lake subbasin, Nevada: 1. Sequence of formation and elevational distribution of carbonate deposits (tufas). Palaeogeography, Palaeoclimatology, Palaeoecology, 109(1), 55-87.
Durrast, H., Siegesmund, S. (1999). Correlation between rock fabrics and physical properties of carbonate reservoir rocks. Journal Earth Sciences 88, 392-408
Engelder, T., & Marshak, S. (1985). Disjunctive cleavage formed at shallow depths in sedimentary rocks. Journal of Structural Geology, 7(3-4), 327-343.
Enos, P., & Sawatsky, L.H. (1981). Pore networks in Holocene carbonate sediments. Journal of Sedimentary Research, 51(3), 961-985.
Fall, A., & Bodnar, R. J. (2018). How precisely can the temperature of a fluid event be constrained using fluid inclusions?. Economic Geology, 113(8), 1817-1843.
Fall, A., Eichhubl, P., Bodnar, R.J., Laubach, S.E., & Davis, J.S. (2015). Natural hydraulic fracturing of tight-gas sandstone reservoirs, Piceance Basin, Colorado. GSA Bulletin, 127(1-2), 61-75.
Fletcher, R. C., & Pollard, D. D. (1981). Anticrack model for pressure solution surfaces. Geology, 9(9), 419-424.
Gale, J.F.W., Lander, R.H., Reed, R.M., & Laubach, S.E. (2010). Modeling fracture porosity evolution in dolostone. Journal of Structural Geology, 32(9), 1201–1211.
Garland, J., Neilson, J.E., Laubach, S.E. & Whidden K. J. (2012). Advances in carbonate exploration and reservoir analysis. In Garland, J., J.E. Neilson, S.E. Laubach, & K.J. Whidden (eds.) Advances in Carbonate Exploration and Reservoir Analysis, Geological Society of London Special Publications 370, 1-15. doi: 10.1144/SP370.15
Gillespie, P. A., Johnston, J. D., Loriga, M. A., McCaffrey, K. J. W., Walsh, J. J., & Watterson, J. (1999). Influence of layering on vein systematics in line samples. Geological Society, London, Special Publications, 155(1), 35-56.
Goldstein, R.H. (1986). Reequilibration of fluid inclusions in low-temperature calcium-carbonate cement. Geology, 14(9), 792-795.
Laubach, S.E., Lander, R.H., Criscenti, L.J., et al. (2019). The role of chemistry in fracture pattern development and opportunities to advance interpretations of geological materials. Reviews of Geophysics, 57 (3), 1065-1111. doi:10.1029/2019RG000671
Laubach, S.E., Fall, A., Copley, L.K., Marrett, R., & Wilkins, S.J. (2016). Fracture porosity creation and persistence in a basement-involved Laramide fold, Upper Cretaceous Frontier Formation, Green River Basin, USA. Geological Magazine, 153(5-6), 887-910.
Laubach, S.E., Olson, J.E., & Gross, M.R. (2009). Mechanical and fracture stratigraphy. AAPG Bulletin, 93(11), 1413-1426.
Liu, L.H., Ma, Y.S., Liu, B., & Wang, C.L. (2017). Hydrothermal dissolution of Ordovician carbonates rocks and its dissolution mechanism in Tarim Basin, China. Carbonates and Evaporites, 32(4), 525-537.
Lucia, F.J. , & Major, R.P. (1994). Porosity evolution through hypersaline reflux dolomitization. Dolomites: A Volume in Honour of Dolomieu, 325-341.
Narr, W., Schechter, D.S., & Thompson, L.B. (2006). Naturally fractured reservoir characterization (112). Richardson, Texas: Society of Petroleum Engineers.
Nelson, R.A., Serra, S. (1995). Vertical and lateral variations in fracture spacing in folded carbonate sections and its relation to locating horizontal wells. Journal of Canadian Petroleum Technology. 34 (6).
Ortega, O.J., Gale, J.F., & Marrett, R. (2010). Quantifying diagenetic and stratigraphic controls on fracture intensity in platform carbonates: An example from the Sierra Madre Oriental, northeast Mexico. Journal of Structural Geology, 32(12), 1943-1959.
Ruggieri, G., & Gianelli, G. (1999). Multi-stage fluid circulation in a hydraulic fracture breccia of the Larderello geothermal field (Italy). Journal of Volcanology and Geothermal Research, 90(3-4), 241-261.
Silva, O.L., Bezerra, F.H., Maia, R.P., & Cazarin, C.L. (2017). Karst landforms revealed at various scales using LiDAR and UAV in semi-arid Brazil: Consideration on karstification processes and methodological constraints. Geomorphology, 295, 611-630.
Smith Jr, L.B., & Davies, G.R. (2006). Structurally controlled hydrothermal alteration of carbonate reservoirs: Introduction. AAPG Bulletin, 90(11), 1635-1640.
Ukar, E. and Laubach, S.E. (2016). Syn- and postkinematic cement textures in fractured carbonate rocks: Insights from advanced cathodoluminescence imaging, Tectonophysics 690, Part A, 190-205, doi: 10.1016/j.tecto.2016.05.001
Zahm, C.K., Zahm, L.C., & Bellian, J.A. (2010). Integrated fracture prediction using sequence stratigraphy within a carbonate fault damage zone, Texas, USA. Journal of Structural Geology, 32(9), 1363-1374.
© 2024 Jackson School of Geosciences, The University of Texas at Austin